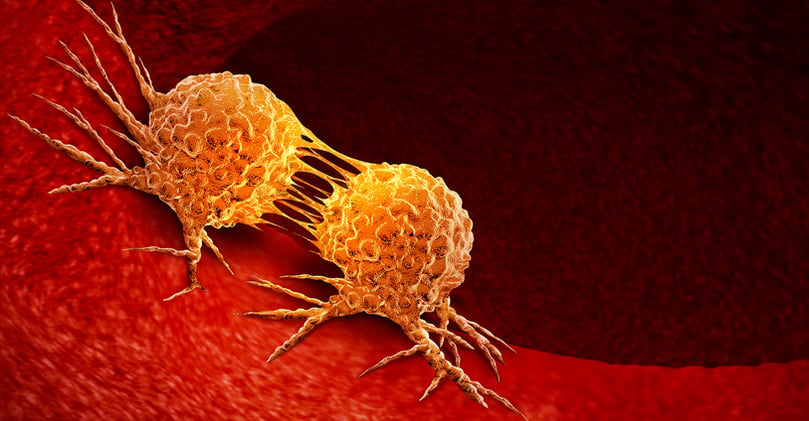
Human epidermal growth factor receptor 2 (HER2; ERBB2) has been shown to induce oncogenesis in several cancers, particularly breast and gastric cancers. HER2 is estimated to be overexpressed in ~20% of all breast cancers, and outcomes for these breast cancers were poor before HER2-targeted therapies[1]. Our understanding of mechanisms by which HER2 drives tumor growth and metastasis led to one of the first targeted therapies for treating HER2+ breast cancers. Here we highlight what is known about HER2 and oncogenesis. and how this information can be applied to the development of new targeted therapies.
Gene amplification of the ERRB2 oncogene is one of the most common genetic defects detected in HER2+ tumors and typically causes overexpression of HER2 at the cell membrane[2]. HER2 proteins more readily dimerize with other HER2 proteins to form homodimers or with other ERRB members to form heterodimers. These complexes induce oncogenic signaling cascades, including MAPK and PI3K/AKT/mTOR, which cause cellular proliferation, angiogenesis, and resistance to apoptosis[3]. Due to the dominant role of HER2 in breast cancer, anti-HER2 antibodies were developed as one of the first targeted therapies. In 2006, clinical trial results of breast cancer patients treated with the anti-HER2 antibody trastuzumab showed significant improvements in progression-free survival and overall survival[4],[5]. Unfortunately, ~20-25% of metastatic breast cancer patients develop resistance to anti-HER2 therapies. More recent studies have focused on engineering new forms of anti-HER2 antibodies or developing HER1/2 inhibitors that can be used alone or in combination with other targeted therapies.
The current standard of care for HER2+ breast cancer includes chemotherapy with adjuvant HER2-targeted therapies and endocrine therapy as indicated. Advances in targeted therapies are further improving treatment protocols. The next-generation anti-HER2 monoclonal antibody margetuximab targets the same HER2 epitope as trastuzumab and is modified in its Fc domain to improve antibody-dependent cellular cytotoxicity.[6] Margetuximab was approved by the FDA in December 2020 for the treatment of metastatic breast cancer in patients who had received prior HER2 therapies[7]. Two different bispecific antibodies have also been developed and are in clinical trials: ZW25 is a bispecific antibody that targets two different HER2 epitopes, and PRS-343 is a bispecific antibody that targets a HER2 epitope and the 4-1BB costimulatory immunoreceptor[8]. Bispecific antibodies are under development for breast cancer and other cancers because they exploit the property that antibodies have two epitope binding sites and can be engineered to better target a single molecule or crosslink multiple molecules, like HER2 and immune checkpoint molecules.
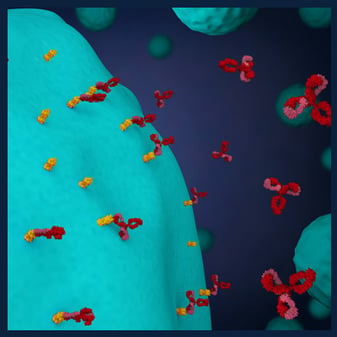
Antibody-drug conjugates have also been evaluated as novel therapeutics that target HER2. Trastuzumab deruxtecan is an anti-HER2 antibody conjugated to a cytotoxic payload that is linked by a cleavable drug linker[9]. This treatment has been recently approved by the FDA and it shows superior targeting of cytotoxic drugs to HER2+ tumor cells and shows efficacy on individuals who have been treated previously with anti-HER2 mAbs.
HER2-specific tyrosine kinase inhibitors have also been successful in treating HER2+ tumors, especially brain metastases because these drugs can cross the blood-brain barrier. Lapatinib, neratinib, pyrotinib, and tucatinib are four HER2-specific tyrosine kinase inhibitors used to treat HER2+ breast cancers. Lapatinib is an oral, irreversible small molecule HER1/HER2/HER4 TKI that was first approved in 2007 for the treatment of metastatic breast cancer[10]. Neratinib is a similar, orally available irreversible small molecule HER1/HER2/HER4 TKI that showed better efficacy against EGFR mutants and has been approved for use as an adjuvant therapy following trastuzumab treatment[11]. The development of resistance is also a problem for HER2-targeted TKIs, and pyrotinib is an experimental HER1/HER2/HER4 TKI under investigation as a potential new drug that is less prone to resistance[12]. Tucatinib is a TKI that is highly selective for HER2 approved for use in combination with trastuzumab and capecitabine in HER2+ breast and colorectal cancers, including patients with metastases and who have received previous anti-HER2 treatment[13].
HER2+ breast cancers continue to be challenging to treat due to resistance and metastasis. Combination therapies with antibodies and targeted inhibitors have dramatically improved survival in HER2+ breast cancer patients, but individuals who relapse continue to be faced with limited treatment options. New antibody-drug conjugates, bispecific antibodies, and targeted inhibitors are under investigation and hold the promise of better, more durable treatment options for patients.

[1] Hudis CA. Trastuzumab--mechanism of action and use in clinical practice. N. Engl. J. Med. 2007 Jul 5;357(1):39-51.
[2] Slamon DJ, Clark GM, Wong SG, Levin WJ, Ullrich A, McGuire WL. Human breast cancer: correlation of relapse and survival with amplification of the HER-2/neu oncogene. Science. 1987 Jan 9;235(4785):177-82.
[3] Moasser MM. The oncogene HER2: its signaling and transforming functions and its role in human cancer pathogenesis. Oncogene. 2007 Oct 4;26(45):6469-87.
[4] Romond EH, Perez EA, Bryant J, Suman VJ, Geyer Jr CE, Davidson NE, Tan-Chiu E, Martino S, Paik S, Kaufman PA, Swain SM. Trastuzumab plus adjuvant chemotherapy for operable HER2-positive breast cancer. N. Engl. J. Med. 2005 Oct 20;353(16):1673-84.
[5] Smith I, Procter M, Gelber RD, Guillaume S, Feyereislova A, Dowsett M, Goldhirsch A, Untch M, Mariani G, Baselga J, Kaufmann M. 2-year follow-up of trastuzumab after adjuvant chemotherapy in HER2-positive breast cancer: a randomised controlled trial. Lancet. 2007 Jan 6;369(9555):29-36.
[6] Rugo HS, Im SA, Cardoso F, Cortés J, Curigliano G, Musolino A, Pegram MD, Wright GS, Saura C, Escrivá-de-Romaní S, De Laurentiis M. Efficacy of margetuximab vs trastuzumab in patients with pretreated ERBB2-positive advanced breast cancer: a phase 3 randomized clinical trial. JAMA Oncol. 2021 Apr 1;7(4):573-84
[7] https://www.fda.gov/drugs/resources-information-approved-drugs/fda-approves-margetuximab-metastatic-her2-positive-breast-cancer.
[8] Kunte S, Abraham J, Montero AJ. Novel HER2-targeted therapies for HER2-positive metastatic breast cancer. Cancer. 2020 Oct 1;126(19):4278-4288.
[9] Doi T, Shitara K, Naito Y, Shimomura A, Fujiwara Y, Yonemori K, Shimizu C, Shimoi T, Kuboki Y, Matsubara N, Kitano A, Jikoh T, Lee C, Fujisaki Y, Ogitani Y, Yver A, Tamura K. Safety, pharmacokinetics, and antitumour activity of trastuzumab deruxtecan (DS-8201), a HER2-targeting antibody-drug conjugate, in patients with advanced breast and gastric or gastro-oesophageal tumours: a phase 1 dose-escalation study. Lancet Oncol. 2017 Nov;18(11):1512-1522.
[10] Ryan Q, Ibrahim A, Cohen MH, Johnson J, Ko CW, Sridhara R, Justice R, Pazdur R. FDA drug approval summary: lapatinib in combination with capecitabine for previously treated metastatic breast cancer that overexpresses HER-2. Oncologist. 2008 Oct;13(10):1114-9.
[11] Minami Y, Shimamura T, Shah K, LaFramboise T, Glatt KA, Liniker E, Borgman CL, Haringsma HJ, Feng W, Weir BA, Lowell AM, Lee JC, Wolf J, Shapiro GI, Wong KK, Meyerson M, Thomas RK. The major lung cancer-derived mutants of ERBB2 are oncogenic and are associated with sensitivity to the irreversible EGFR/ERBB2 inhibitor HKI-272. Oncogene. 2007 Jul 26;26(34):5023-7.
[12] Li Q, Guan X, Chen S, Yi Z, Lan B, Xing P, Fan Y, Wang J, Luo Y, Yuan P, Cai R, Zhang P, Li Q, Zhong D, Zhang Y, Zou J, Zhu X, Ma F, Xu B. Safety, Efficacy, and Biomarker Analysis of Pyrotinib in Combination with Capecitabine in HER2-Positive Metastatic Breast Cancer Patients: A Phase I Clinical Trial. Clin. Cancer Res. 2019 Sep 1;25(17):5212-5220.
[13] Murthy RK, Loi S, Okines A, Paplomata E, Hamilton E, Hurvitz SA, Lin NU, Borges V, Abramson V, Anders C, Bedard PL, Oliveira M, Jakobsen E, Bachelot T, Shachar SS, Müller V, Braga S, Duhoux FP, Greil R, Cameron D, Carey LA, Curigliano G, Gelmon K, Hortobagyi G, Krop I, Loibl S, Pegram M, Slamon D, Palanca-Wessels MC, Walker L, Feng W, Winer EP. Tucatinib, Trastuzumab, and Capecitabine for HER2-Positive Metastatic Breast Cancer. N Engl J Med. 2020 Feb 13;382(7):597-609.